Generating Power on Earth From the Coldness of Deep Space
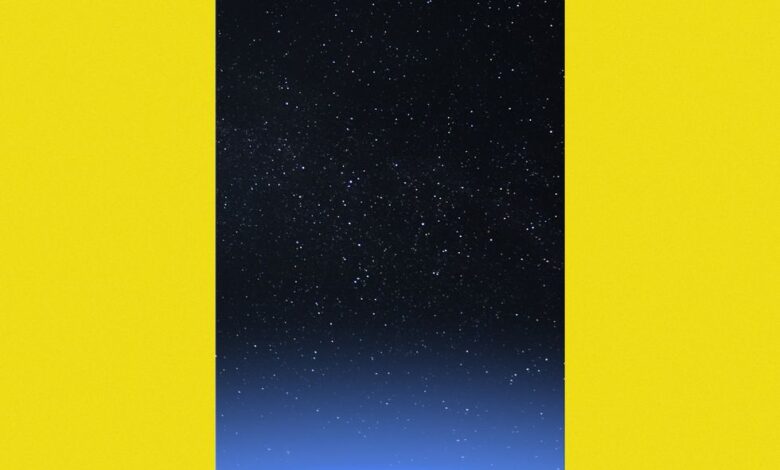
It’s a summer night. On the rooftop of a quiet building, a set of panels cools the rooms within and keeps the lights on, removing heat and generating electricity using the coldness of the sky. That cold isn’t in the air around the building—the night is warm. Rather, the panels reach far beyond Earth’s atmosphere to tap the distant cold of deep space.
Sound crazy? Admittedly, this technology isn’t fully available just yet. But we have demonstrated that by directly using power generated by the cold universe, we can chill water to cool buildings by as much as 5 ºC during the day without electricity and light the night without wires or batteries. As the technology improves, we see it enabling solar panels that work at night as well as day, powering remote sensors.
From the time the first humans learned to harness fire, people have manipulated heat to do their bidding. Today, the art of turning heat from burning gas, nuclear fission, Earth’s core, the sun, and other sources into useful energy underpins modern life.
Edmon de Haro
With so much energy available from heat, we’ve ignored another source of power: cold. The coldness of deep space is a thermodynamic resource, and largely untapped. Yes, it’s far away, but distance doesn’t prevent its use, particularly when we consider just how cold the vast empty space of the universe is—approximately 3 kelvins.
We generally aren’t aware of this coldness because things around us, including sunlight and radiation bouncing back to us from the atmosphere, conspire to heat us up. But about a decade ago, our research group at Stanford designed a material that’s remarkably efficient at sending heat out to that reservoir of cold while preventing heating from both the sun and the environment. The material is so efficient, in fact, that it can cool itself below the temperature of its surroundings, even when sitting in direct sunlight.
That was pretty cool—literally. And when heat can spontaneously flow from an object on Earth to the universe, just like water flows from higher ground to the sea, it gives us an opportunity to harvest useful energy from it along the way.
In the case of moving water, a turbine harvests the energy in the flow to generate hydroelectricity. In the case of the flow of heat from Earth to deep space, we’ve got a couple of promising concepts developed, although we’re still trying to figure out the best mechanism.
Thermodynamics on Earth and in space
Before we tell you about those ideas and prototypes, you need to understand the role radiation plays in maintaining Earth’s energy balance.
Radiation is one of three mechanisms for heat transfer. The other two are heat conduction and heat convection. The first arises from atoms vibrating against one another as typically occurs in a solid; the second arises from bulk movements of particles, such as gas molecules in air. Both conduction and convection require a medium through which to move heat. Radiation, in the form of traveling electromagnetic waves, does not require such a medium and can traverse a long distance.
Consider solar radiation, which carries heat from the sun to Earth’s surface. On a sunny day, you can feel your body heat up as it absorbs that sunlight. Earth-based objects radiate heat, too: On a clear night you’ll feel your body cool; some of that cooling is heat radiating into space.
While incoming radiation has become a mainstay for renewable energy in the form of solar energy, outgoing radiation has largely remained untapped for energy generation. That outgoing radiation sends the heat from an object on Earth to outer space, a reservoir with virtually limitless capacity. Removing heat this way can cool that object down tens of degrees below the temperature of its surroundings.
We can exploit the temperature difference by turning it into electricity through thermoelectric power generation. The working principle behind a thermoelectric generator is the Seebeck effect, which describes how a material develops a voltage difference in response to a temperature differential across it. We can manipulate the Seebeck effect in semiconductors by the controlled addition of impurities, or dopants.
Recall that dopants can turn their host semiconductors into either n-type semiconductors, with mobile negatively charged electrons, or p-type semiconductors, with mobile positively charged holes. In either case, when these semiconductors bridge a temperature differential, the electrons or the holes congregate near the colder end. So the n-type develops a positive voltage potential toward the hot side, while the p-type develops a negative voltage potential in the same direction.
A thermoelectric generator (TEG) consists of alternating pairs of n– and p-type semiconductors chained together so that the voltage gained from the positive temperature differential in an n-type adds to the voltage gained from the negative temperature differential in a p-type. By connecting a TEG between a hot reservoir and a cold one, the heat differential is captured as electricity.
With the ambient environment as a hot reservoir, we can use the coldness from deep space to create the cold reservoir.
To do this, we send heat out to space using what we call an emitter, which cools itself to a lower temperature than its surroundings. That’s a phenomenon known as radiative cooling. Then, a thermoelectric generator situated between the cold emitter and the now-hotter ambient surroundings can produce electricity.
The emitter’s job is to radiate the heat out beyond Earth’s atmosphere. But the atmosphere is transparent only to photons of certain wavelengths. Within the mid-infrared range, which is where heat radiation from typical earthbound objects is concentrated, the most applicable atmospheric transmission band is in the 8- to 13-micrometer-wavelength range.
Even some simple emitters send out heat radiation at these wavelengths. For example, if it’s insulated from ambient surroundings, black paint emits enough radiation within that band to cool a surface down by 10 ºC when exposed to the night sky.
In the wavelength range outside 8 to 13 mm, the atmosphere bounces back a substantial amount of radiation. During the daytime, solar radiation comes into the equation. More-advanced emitter designs aim to avoid the incoming radiation from the atmosphere and sunlight by ensuring that they absorb and emit only within the transparency window. The idea of using such a wavelength-selective emitter for radiative cooling dates back to the pioneering work of Claes-Göran Granqvist and collaborators in the 1980s. Just as an engineer designs a radio antenna with a specific shape and size to transmit over a certain wavelength in a certain direction, we can design an emitter using a library of materials, each with a specific shape and size, to adjust the wavelength band and direction for heat radiation. The better we do this, the more heat the emitter ejects into space and the colder the emitter can get.
Glass is a great material for an emitter. Its atomic vibrations couple strongly to radiation around the 10-μm wavelength, forcing the material to emit much of its heat radiation within the transmission window. Just touch a glass window at night and you’ll feel this cooling. Adding a metallic film to help reflect radiation skyward makes the emissions—and the cooling—even more effective. And structures can be specifically designed to strongly reflect the wavelengths of sunlight.
When an emitter radiates heat at a wavelength within the atmospheric transmission window, it cools down, creating a cold reservoir. A thermoelectric generator can then use the ambient air as its hot side and the emitter as its cold side to produce electricity. Chris Philpot
A decade ago, our research group created the first radiative cooling material that works in the daytime, efficiently cooling itself down below the ambient air temperature, even in direct sunlight. It’s constructed from alternating thin films of hafnium oxide (HfO2) and glass sitting on top of a silver reflective layer. By carefully selecting the thicknesses of each layer of film, we were able to make this material reflect solar radiation almost completely while simultaneously sending heat out through the atmospheric transmission window.
Since then, many other research groups have demonstrated various designs for daytime radiative cooling. One group of researchers at the University of Colorado, Boulder, designed an emitter by embedding a polymer film with microscopic glass beads and coating the back of it with a thin layer of silver. The glass beads send heat radiation out from the polymer while the silver coating reflects incoming sunlight.
As for our material, we have already commercialized one application: cooling structures without the use of electricity, thereby reducing or eliminating the need for building air-conditioning. SkyCool Systems, a spin-off from our research group, sells passive cooling panels that can be used as a stand-alone cooling system or as an add-on to existing air-conditioning and refrigeration systems. So far, SkyCool has installed panels at a number of grocery stores across the United States.
Harvesting cold for energy harvesting
In a 2017 proof of concept, replicated in November 2023 [top], the emitter is a black-painted aluminum plate inside an insulation chamber whose plastic cover is transparent to mid-infrared radiation. A thermoelectric generator inserted in the bottom of the chamber uses the emitter as its cold source and the metal stand as its heat source to power an LED. In a later experiment [bottom], a solar cell serves as the emitter. During the daytime, the solar cell generates electricity from sunlight. At the same time, the thermoelectric generator produces extra electricity from the heat flowing between the solar cell and its colder surroundings. At night, the generator produces electricity from the opposite heat flow—between the hotter surroundings and the colder emitter.Photos: Sid Assawaworrarit/Stanford University
Energy harvesting using the cold of the universe is still under development. As our first proof of concept, we made a simple emitter using black paint on an aluminum plate. We enclosed the emitter in a foam box with a cover of transparent polyethylene film; this allowed the emitter to radiate heat into space while insulating it against heat from the surroundings.
We then cut a small hole in the bottom of the foam box and attached an off-the-shelf thermoelectric generator to the emitter (which you’ll recall also acts as a cold sink). For the hot side of our generator, we attached a heat sink that passively collected heat from the immediate surroundings.
To avoid having to contend with sunlight, we tested this setup at night, on the rooftop of Stanford’s David Packard Electrical Engineering Building. It generated 25 milliwatts of power per square meter of our emitter’s surface area and lit up an LED.
Our system resembled a solar panel, so we began to consider the possibilities of combining the two technologies for a device that generates power day and night. Commercial silicon solar cells typically have a top protective layer made of silica glass, which transmits a significant amount of heat radiation at the frequencies needed to traverse the atmosphere. Using that glass as the emitter, with a similar insulation setup as our first demonstration and a thermoelectric generator inserted between the glass and the solar cell, we demonstrated 50 milliwatts per square meter of nighttime electricity generation, without interrupting the photovoltaic’s daytime functioning.
While interesting, a 50 mW/m2 power density is of little practical use; even a suburban grocery store rooftop—say, about 4,000 m2—would yield just 200 watts, about enough to power a small refrigerator. We needed to increase the power density of our energy harvester to make it an attractive option for powering lighting and other low-power electronics at night. So we began testing modifications to our setup in a simulated model, discovering a number of ways to improve our design.
The key is optimizing the size of the thermoelectric generator for a given emitter area. A larger generator produces more power for a given degree of temperature difference between the emitter and the ambient surroundings, but it lowers the temperature difference that the emitter can sustain by permitting more heat to flow between the two. By getting the balance right, we demonstrated a doubling of power density to more than 100 mW/m2, using just the black-paint emitter.
Thermally insulating the emitter from its surroundings to allow it to reach a very cold temperature is also very important. Obviously, much better insulating materials are available than those used in our demo.
Finally, more spectrally selective emitters, like the glass-bead design and the multilayer hafnia design described, cool to much lower temperatures than black paint on aluminum, and therefore increase the power density.
Putting all these optimizations together, we calculated that the maximum achievable power density for this technology is 2.2 W/m2. This power density is a lot lower than what can be generated with solar cells under sunlight. However, when sunlight isn’t readily available, this is pretty good; it’s significantly higher compared to what can be achieved with many other ambient energy-harvesting schemes. For example, it’s orders of magnitude more than the less than 1 mW/m2 that can be harvested from ambient radio waves.
Our approach here hinges on using the emitter to both send out heat radiation to cold space and act as a local cold reservoir. That means we must insulate the emitter to prevent a constant intrusion of heat to maintain the temperature difference.
But what if we didn’t need that local temperature difference to generate electricity? To answer this question, we looked to solar photovoltaics, to determine if there is a cold analog that works with deep space instead of sunlight.
A photovoltaic cell can generate electricity from both the absorption and the emission of heat radiation. When the cell is exposed to heat radiation from a hotter body, a large number of electron-hole pairs form, and the cell develops a positive voltage potential. When the cell is exposed to a colder body, electrons and holes in the cell recombine into outgoing radiation, and the cell develops a negative voltage potential.Chris Philpot
The negative solar cell
In solar energy harvesting, a photovoltaic cell generates electricity directly from the sun’s radiation, thanks to what happens inside a semiconductor as it absorbs light. Recall that electrons and holes—the charge carriers in a semiconductor—normally exist in a minute quantity in an undoped semiconductor, as a result of thermal excitation at room temperature. But if you bombard the semiconductor with photons having energies greater than the bandgap of the semiconductor, you can generate many more electrons and holes. To separate the photogenerated electrons and holes, selective contacts—those that allow only one type of charge carrier to pass through—are attached to both sides of the semiconductor. A common way to do this is to dope one side of the semiconductor so that it’s p-type, which lets holes pass and blocks electrons, and the other side so that it’s n-type, which lets electrons pass and blocks holes. The result is an accumulation of holes on the p-side and electrons on the n-side, giving the p-side a positive voltage relative to the n-side; electrons flow from the n-side when a load is connected.
This familiar picture of photovoltaic operation assumes a relatively cold photovoltaic cell on Earth bathed in bright radiation coming from a much hotter body like the sun. The cold analogue is a photovoltaic cell on Earth facing the void of space. Here, Earth is hot compared to space, and the temperature difference means that the earthbound photovoltaic cell emits net radiation to space.
In such a case, the electrons and holes in the semiconductor recombine and radiate photons, reversing the process of light absorption. This recombination eats up the population of electrons and holes, pulling holes away from the p-side and electrons away from the n-side. With no incoming radiation to balance the radiative recombination, the depopulation of charges on both ends causes the p-side to develop a negative voltage relative to the n-side. Connect a load and electrons flow from the p-side. The voltage polarity is the opposite of the scenario in which a cold photovoltaic cell absorbs radiation from the hot sun—but it’s still electricity. This phenomenon of a solar cell generating energy when facing a cold object is not surprising; it is implied in the well-known Shockley-Queisser limit, which explains the maximum theoretical efficiency of a solar cell.
More recently, our research group and others studied the possibility of using such a device to harvest electricity from the heat radiation that Earth releases to the universe. We call this “negative” illumination for its net release of radiation, to distinguish it from the “positive” illumination that occurs in a solar cell. Some others call it thermoradiative energy harvesting.
To make negative illumination work for energy harvesting on Earth requires the photovoltaic cell to emit radiation at a wavelength within the atmospheric transmission window. In this window, the electrons and holes can recombine into outgoing radiation. Outside the window, the radiation bouncing back from the atmosphere destroys the process that creates that negative voltage. To hit that transmission window, we have to create the photovoltaic cell from a semiconductor with a tiny bandgap—around 0.09 electron volts—which corresponds to the edge of the transmission window at a wavelength of 13 μm.
That’s indeed possible, though not with silicon. In our first laboratory experiment, we used a mercury cadmium telluride (MCT) photovoltaic cell with a bandgap of around 0.1 eV. We confirmed the negative illumination effect by pointing the MCT cell at a temperature-controlled surface. The setup allowed us to heat up the surface to make it emit more radiation—allowing our MCT cell to operate under positive illumination—and then to cool down the surface, allowing the MCT cell to switch to negative illumination. By changing the temperature of the surface, we were able to observe the transition between positive illumination and negative illumination from the corresponding change in the cell’s voltage output.
We then took our MCT cell out of the lab and pointed it at the night sky to test the effect using the cold universe. We did generate electricity, but at a power density of just 64 nanowatts per square meter, much lower than that of our emitter-based approach.
A couple of things were to blame. First, the bandgap of the MCT cell is just a little too high to be in the ideal transmission window. Second, small bandgap semiconductors suffer greatly from nonradiative processes—that is, electron-hole recombinations that don’t emit radiation. Combined, these reduced the power our cell could deliver.
Pushing the technology into the future
In an almost perfect world, in which we have discovered the best materials for emitters and negative-illumination photovoltaic cells and solved all our other design problems, we calculate that the maximum power density for the thermoelectric emitter system and the negative illumination approaches is around 5 W/m2. That’s about one-thirtieth what commercial solar cells deliver at peak sunlight or about the same as what a solar cell produces inside a brightly lit office.
In a more realistic scenario, we think we can reach a power density on the order of 1 W/m2. That may not sound like much, but it’s sufficient to power LED lighting and air-quality sensors, and keep smartphone batteries charged. In the long run, it’s perhaps not unreasonable to imagine living in a faraway cabin, off the grid, without batteries, using incoming and outgoing radiation from far beyond Earth’s atmosphere to heat, cool, and generate electricity day and night.
IEEE Spectrum